Demystifying Loop Quantum Gravity: A Dive into the Quantum Fabric of the Universe
Amid the towering achievements and persistent mysteries of modern physics, one theory attempts to reconcile the monumental pillars of general relativity and quantum mechanics: Loop Quantum Gravity (LQG). As an avid enthusiast of physics, particularly quantum field theory, the exploration of the universe at its most fundamental level has always fascinated me. Loop Quantum Gravity, with its audacious aim to describe the quantum fabric of space-time itself, represents a thrilling frontier in theoretical physics. In this article, we will delve into the core concepts behind Loop Quantum Gravity, its implications, and why it stands out as a promising candidate for a theory of quantum gravity.
Understanding Space-Time as Quantized
At the heart of Loop Quantum Gravity is the concept that space-time is not a continuous fabric but consists of finite, discrete quanta. This concept fundamentally shifts our understanding from the smooth space-time described by Albert Einstein’s general relativity to a granular picture at the Planck scale (about \(10^{-35}\) meters).
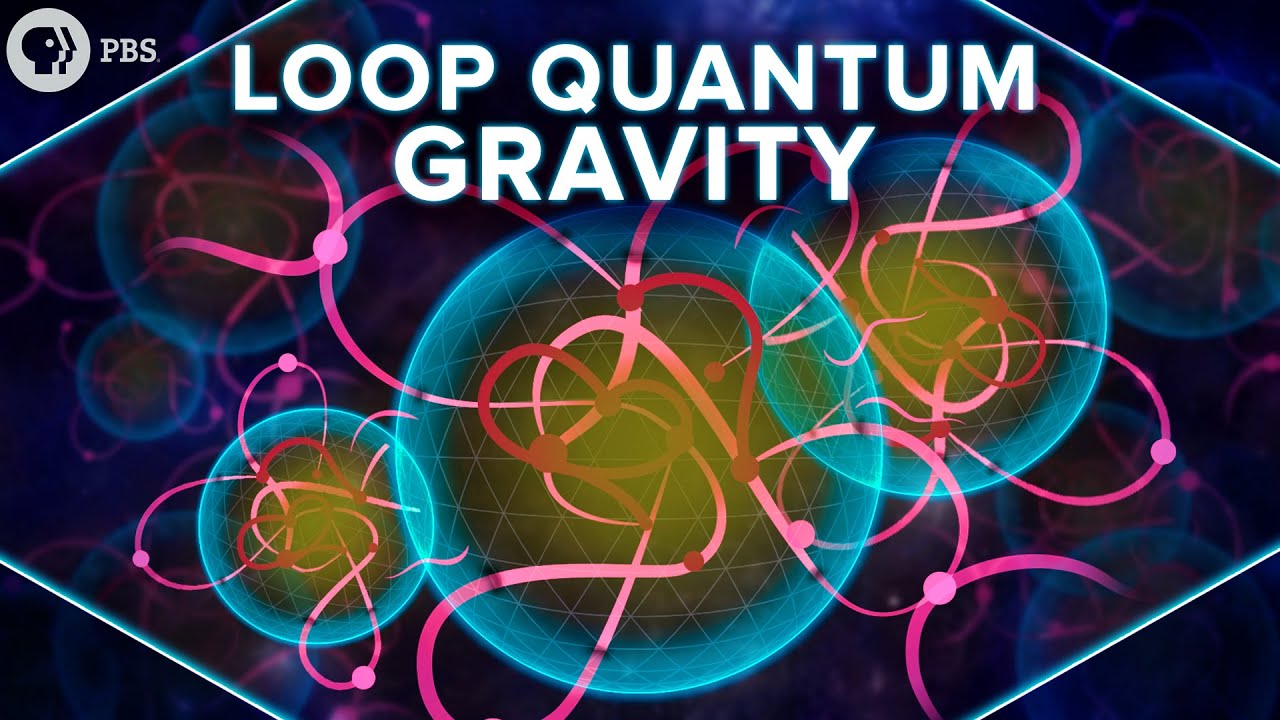
LQG mathematically formulates this quantum space-time using what are termed “spin networks” – abstract graphs whose edges and nodes embody the quantum states of space-time geometry. These networks evolve over time, depicting a dynamic, quantized geometry that differs markedly from the static, continuous field of classical relativity.
The Role of Spin Networks and Spin Foams
Spin networks provide the skeleton of space in LQG, offering a snapshot of the quantum state of the gravitational field. Nodes within these networks represent quanta of space, while the edges depict the spatial relationships between them. Transitioning from one quantum state to another, spin networks evolve, thereby generating a history of geometrical changes. This history is chronicled by “spin foams”, which are the higher-dimensional analogs of spin networks, representing space-time’s quantum evolution.
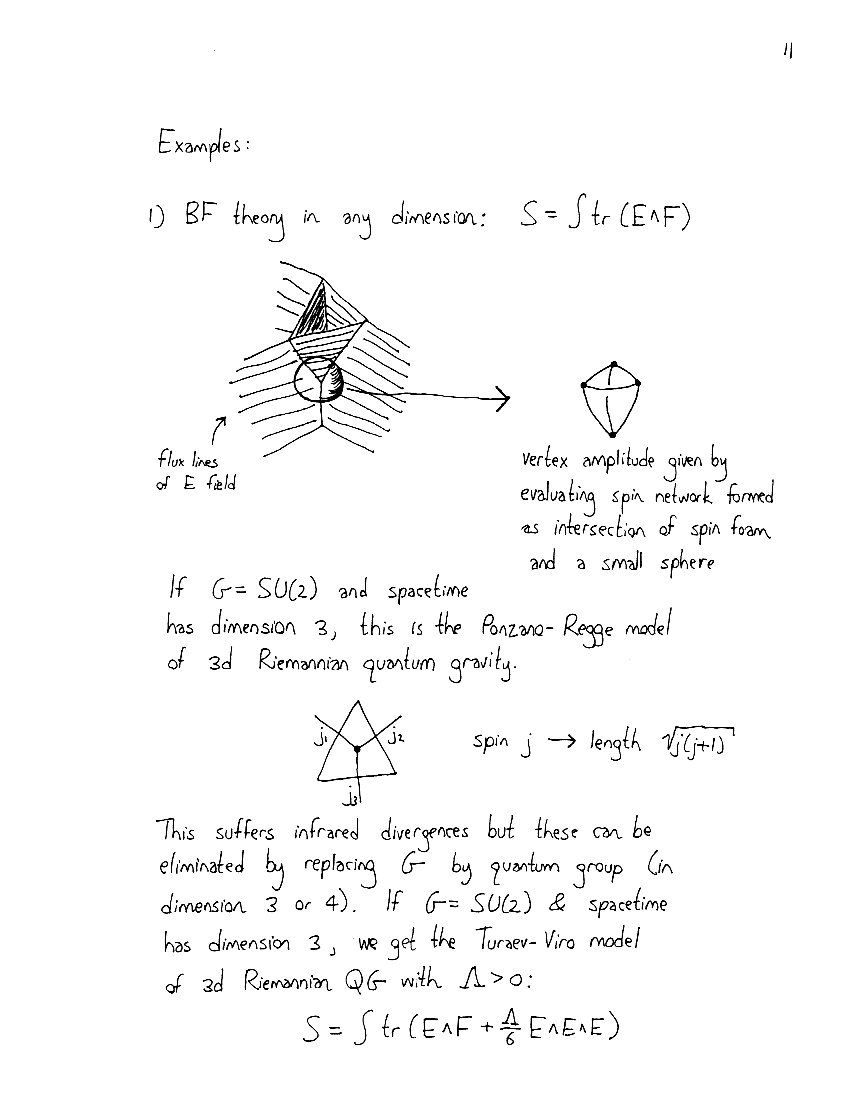
Through spin networks and spin foams, LQG introduces a quantum theory that is background-independent, meaning it does not presuppose the existence of a fixed space-time backdrop, aligning with the principle of general relativity that space-time’s geometry is dynamic.
Implications for Black Hole Physics and Early Universe Cosmology
Loop Quantum Gravity’s quantized vision of space-time has profound implications, particularly in the realms of black hole physics and the universe’s infancy. By applying LQG to these extreme conditions, researchers have derived tantalizing insights, such as the possibility that black holes have discrete, quantized areas. This quantization could potentially solve the black hole information paradox, suggesting information could be preserved rather than destroyed by black holes.
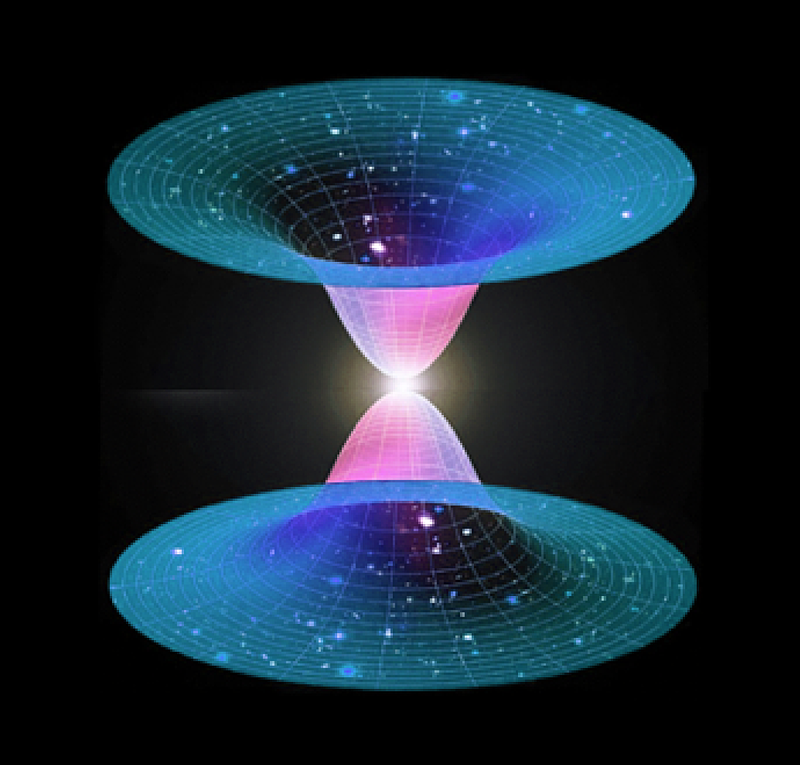
In early universe cosmology, LQG offers a glimpse into pre-Big Bang scenarios, proposing mechanisms that could explain the universe’s bounce from a previous, contracting phase to its current expansion, avoiding the classical singularity problem entailed by the Big Bang theory.
Challenges and the Path Forward
Despite its promising prospects, Loop Quantum Gravity faces significant challenges. Notably, deriving tangible predictions that can be tested experimentally has proven difficult, a hurdle that must be overcome to empirically validate the theory. Furthermore, reconciling LQG with the vast array of phenomena explained by quantum field theory and the Standard Model of particle physics remains an ongoing endeavor.
In conclusion, as we navigate this intricate dance of quantum mechanics and general relativity, Loop Quantum Gravity offers a compelling framework that challenges our conventional understanding of the universe. Its exploration embodies the essence of physics and philosophy – an unending quest to comprehend the cosmos’s fundamental nature. As someone deeply enamored by the mysteries of physics, following the advancements in Loop Quantum Gravity feels like participating in an epoch-defining journey, one that inches us closer to unlocking the universe’s ultimate secrets.